Daily Newsletter
October 3, 2013 Respiratory Chain & Fermentation
The metabolic pathways of glycolysis, formation of acetyl CoA, and the citric acid cycle provide the means of harvesting reducing potential from the complete breakdown of glucose. While there are a few substrate level phosphorylation events, the bulk of energy harvested is in the form of reducing potential held by reduced electron carriers. But what happens to these electron carriers.
As with other aspects of metabolism, they can have multiple uses. For example, some biosynthesis reactions will require reducing potential in the form of reduced electron carriers. The bulk of the electron carriers though will be used to power respiratory chains.
In prokaryotes, the respiratory chain will be found on the cell membrane. These respiratory chains will use the reducing potential to power cell membrane activities, such as ion pumps (active transport) and flagellar spinning. They will also use their respiratory chain to power the production of ATP.
The vast majority of eukaryotes, in contrast, do not have repiratory chains embedded in their membrane. Instead, they rely on their mitochondria. Recall that the inner membrane of the mitochondria is prokaryotic in origin. The mitochondria specializes in using reducing potential to power the production of ATP; eukaryotes

Overview
The respiratory chain (aka, the electron transport chain), is found embedded in the inner mitochondrial membrane. These membrane proteins function as electron carriers, but unlike NAD+ and FAD, they are enzymes that utilize reducing potential to pump H+ from the mitochondrial matrix across the inner membrane into the intramembranal space.Therefore, we have reducing potential providing the energy to pump H+; or put another way, primary active transport (directly using energy to pump). The intramembranal space is small, and is being filled with H+, so this region will be acidic. We are also creating a very strong H+ electrochemical gradient across the inner mitochondrial membrane (potential energy). This gradient is called the proton motive force.
Hydrogen will move though open H+ channels back into the matrix (facilitated diffusion). When an ion moves down its electrochemical gradient, across a membrane, work is done (kinetic energy). The mitochondria utlizes this kinetic energy by having the H+ channel complexed with enzymes that make ATP. As H+ comes back into the matrix, the kinetic energy released though the ion's movment is used to make (regenerate) ATP. This is known as the Chemiosmotic Theory (movement of H+ down its electrochemical gradient across a membrane powers ATP synthesis).
Specifics
The electron carriers reduced during the citric acid cycle (which occurs in the mitochondrial matrix) go to NADH Dehyrogenase, also known as complex I of the respiratory (electron transport) chain. The electron pair will be passed to NADH dehydrogenase,
Why is all of this important? Well, why do you need iron in your diet? Why do organisms that have respiratory chains need iron? The main reason is that iron is an excellent reactant in redox reactions. Iron readily moves through different redox states, so is a great mineral to use when trying to move electrons. We also need iron for oxygen transport in the blood, but cells need iron to move electrons. You will hear more about iron-sulfur complexes in higher level classes.
The flavin group is a strong electron acceptor, and makes NADH a flavoprotein. Flavin groups in proteins can have odd reactions, one of the most critical is the donation of an unpaired electron to O2, forming potent reactive oxygen species such as Superoxide (an oxygen free radical) and the peroxide free radical (hydrogen peroxide). Reactive oxygen species have an unpaired electron, and they become potent oxidation agents (they pull electrons from other molecules). They have the ability to disrupt all organic compounds, including biopolymers. The peroxisome (a vesicle microbody found in cells) is responsible for clearing the cell of reactive oxygen species. NOTE: the formation of the free radicals is a natural by-product of flavoproteins such as NADH reductase.
Ubiquinone is important as an intermediate in electron chains. Ubiqunones can move around the inner membrane, connect the stationary transmembranal H+ pumping electrons carriers. In prokaryotes, the quinones act as a pool, and can accept electrons from sources other than NADH. This allows some procaryotes to harvest electrons from reduced minerals (more about that in microbiology).
NOTE: At this academic level, I want you to become familiar with NADH reductase as an example of a transmembranal H+ pump and electron carrier. The rest of the complexes we will cover in more superficial details.
Ubiquinone will then pass the electrons to complex 3, Coenzyme Q-Cytochrome C Reductase. This transmembranal protein accepts electrons from ubiquinone (which is also known as Coenzyme Q, hence the enzyme name). The protein will use the reducing potential to pump H+ into the intramembranal space. The electrons will then be given to Cytochrome C (cytochrome C will be reduced). NOTE: Coenzyme Q (ubiquinone) is also part of the cells defense against free radicals.
Coenzyme Q is considered an Anti-oxidant (due to its redox capabilities). Since ubiquinone is able to move in the lipid portion of the bilayer, it is lipid soluble. In digestion, it is absorbed through the same route as lipids. Can takinig a Coenzyme Q supplement help me? There are some conditions (like Huntington's disease) where Coenzyme Q is recommended, but there is not enough robust clinical data to confirm benefits in other conditions or athletic performance. Do your research before taking supplements. (BTW...what is implied by the word robust in the above sentence?)
Cytochrome C is another mobile electron carrier, but in this case it is water soluble and must move along the surface of the membrane. This protein is contains a Heme group (like in hemoglobin), which carries single electrons. In prokaryotes, Cytochrome C has been shown to have detoxification properties (oxidizing or reducing compounds), and in eukaryotes it plays a part in apoptosis.
Cytochrome C donates electrons to Complex 4, Cytochrome C Oxidase. This is the final transmembranal H+ pump, and the reducing potential is nearly spent in this pumping action. Cytochrome C Oxidase passes these "spent" electrons to oxygen, forming water. Oxygen is thus the FINAL ELECTRON ACCEPTOR. One interesting aspect about Complex 4 is that it uses copper (Cu) for redox reactions.
What about complex 2?
There is a complex 2, but it is not involved when NADH+ + H+ is used. Complex 2, aka Succinate Dehydrogenase, accepts electrons from FADH2, and it is not transmembranal (so no H+ pumping action). This is an interesting enzyme ☺. Do you remember the newletter yesterday about the citric acid cycle? The conversion of succinate to fumarate is handled by Succinate Dehydrogenase! This is the same enzyme! This is another example of the differences between isolated reactions and reactions inside of cells. For the longest time, chemists thought that FAD would have to be an intermediate in the movement of electrons. Modern biochemistry though has shown that the enzyme that handles the oxidation of succinate is part of a complex of enzymes (quantrenary protein structure) that is known as complex II. In the image of the citric acid cycle shown yesterday, the image author used Q instead of FAD to represent the electron carrier. The reason should now be clear...(hint: succinate is oxidazed, and the electrons are used to reduce ubiquinones. The important thing to remember is that the reducing potential that enters here has missed complex I, NADH Dehydrogenase, which is the first H+ pump. This means that reducing potential is only usuable by Complex III and IV.
Each of the transmembranal H+ pumps will move H+ from the matrix to the intramembranal space. This produces a high H+ electrochemical gradient (potential Energy). ATP synthase is another transmembranal protein found in the inner membrane. It acts as a H+ channel (facilitated diffusion). As H+ moves through the channel, toward the matrix, the enzyme is able to produce ATP from ADP and Pi. Since the reducing potential of NADH+ + H+ was used in complex I, III, and IV, it produces more ATP than the reducing potential harvested by complex II (succinate dehydrogenase).
For our purpose, we will round up the actual figures to whole numbers. NADH+ + H+ will allow for the regeneration (synthesis) of 3 ATP, while the reducing potential harvested at Complex II (or historically, FADH2) will yeild 2 ATP. NOTE: These are actually over estimates, but they work to simplify the math.
ATP Totals
What follows is a discussion of the Theoretical Yield of ATP from the catabolism of 1 molecule of Glucose. Note the phrase theoretical yield. Remember that cells have to decide between energy and carbon precursors, so cells rarely make it this high. We are also using whole number estimates of ATP production. An actual cell will have lower ATP yields. Also remember, eukaryotic mitochondria are more efficient than prokaryotes, and prokaryotes have other uses for the proton motive force. Eukaryotic actual yeilds will be higher. These numbers are also for ONE molecule of Glucose.Glycolysis
- 2 ATP used to phosphorylate intermediates
- 4 ATP generated by Substrate Level Phosphorylation
- 2 NAD reduced to NADH+ + H+.
- NOTE: The NADH+ + H+ gained from glycolysis will only produce 2 ATP each. It is the cost of being reduced in the cytoplasm and then having electrons passed to the inner mitochondrial membrane.
- So, the 2NADH+ + H+ will yeild 4 ATP.
- 2 CO2 released.
- 2 NAD reduced to NADH+ + H+.
- through respiratory chain → 6 ATP
- 4 CO2 released.
- 2 GTP → 2 ATP generated by substrate level phosphorylation
- 6 NAD reduced to NADH+ + H+.
- through respiratory chain → 18 ATP.
- 2 QH2 (FADH2)
- through respiratory chain → 4 ATP.
Where did most of the energy come from? Reducing Potential
Where was most of the ATP made and how? ATP synthase, using the proton motive force generated by the respiratory chain.
What metabolic pathway has the highest ATP yield? Citric Acid Cycle.
Fermentation
What happens if the mitochondria runs out of oxygen?Remember, oxygen is the final electron acceptor. Once the electrons have powered the H+ pumps in the inner mitochondrial membrane, they are at low energy. Electrons don't disappear, they have to be given to another atom/molecule. With the mitochondira, Complex IV passes the electrons (and some hydrogen) to Oxygen, thus forming water.
If not replenished, oxygen concentrations in the mitochondria will start to drop. For humans, this will happen when we either can not get enough oxygen through breathing or when our cells are metabolically very active (e.g., a work-out). If we don't have oxygen, then we don't have a final electron acceptor.
When there is no electron carrier, then Complex IV can not get rid of the electrons it holds. It can not accept electrons from Cytochrom C. If Cytochrom C can not reduce something, then it can not accept electrons from Complex III. This means Ubiquinone will not be able to reduce Complex III, and so can't accept electrons from Complex I. Ultimately, this means that complex I can not oxidize NADH + H+.
Is that really a big deal? YES. Remember talking about Flavin and how it can act up, creating free radicals? One trigger for creating free radicals is an imbalance in the expected ratio of NAD+ and NADH + H+. When you get too much NADH + H+ Complex I starts forming free radicals. (NOTE: this is only one outcome...the point is we need to regenerate a quality of NAD+)
When eukaryotic cells start running out of NAD+, Pyruvate Dehydrogenase becomes inactive (think regulation). This means that the cell has to do something to the building concentration of pyruvate (remember...we don't want to build up product...product should always become a new substrate). Pyruvate is moves to a different reaction or pathway.
At this point remember that the trigger for the shift is a loss of NAD+ and a build up of NADH + H+.
In animal cells, protist, and many fungal cells, the pyruvate will be oxidized into Lactate. In some fungi, the pyruvate enters a small pathway that oxidizes and decarboxylates the molecule, resulting in Ethanol (alcohol fermentation).
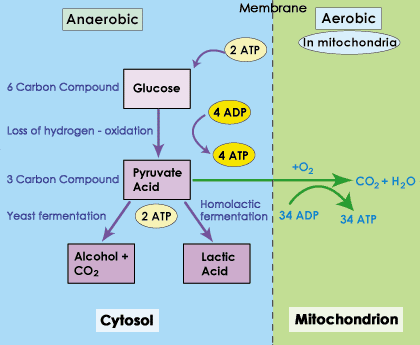
So, what is the result of moving to fermentation. In fermentation, we have to oxidize pyruvate. Electron carriers are needed for redox reactions, so in the oxidation of pyruvate NADH + H+→NAD+. This regenerates NAD+. Since we have NAD+, we can also carry out the substrate level phosphorylations that occur in Glycolysis.
You will recall that the substrate level phosphorylations of glycolysis are not strong energy harvests. More energy is harvested in redox reactions than in substrate level phosphorylation. So, why does it matter that we have some minimal ATP production?
Your cells have critical enzymes that have to keep working for the cell to survive. In an anaerobic state (no oxygen), you don't have enough ATP to carry out all life functions, but there is enough thanks to fermentation to keep critical enzymes running. The goal is then to bring more oxygen into the cells.
Is there a time limit? YES, but it depends on the cell in question. Some fungi can survive in fermentation indefinitely (but they may not be able to survive they're by-product, e.g. alcohol). For other cells, yes, there is a limit. Lactate in water is Lactic Acid, and it does alter pH. More importantly, there are some cells that have more critical function than others. Neurons in the Brain and Heart muscle cells are both very sensitive to low oxygen. Neither can stay in a fermentative state. If these cells do not get oxygen, they will quickly start to die. Other cells can stay ferementative for longer, such as skeletal muscle cells; they will eventually stop working, but they won't die. Once oxygen is restored, the cells will quickly begin to shift back to respiration (aerobic, using the respiratory chain).
What happens to the Lactate? That depends on the organism. Cells will purge themselves of lactate if they can, and will convert some back to pyruvate. There are some cells that are fond of lactate. Heart Muscles actually prefer lactate to glucose, and liver cells can convert lactate back to glucose.
Lactate is not wasted.
At this point it needs to be noted that bacteria have a very different relationship with fermentation. There are some bacteria that live exclusively as fermenters, and others that happily switch between respiration and fermentation. Also, bacteria have many more ways of altering pyruvate than the production of Lactate or Ethanol. Some of these, such as the formation of acetate, have become industrially important.
No comments:
Post a Comment